Lithium-Ion-Batteries are a key technology for green energy, mobility, and for this reason a key technology to mitigate climate change. However, batteries have a significant environmental impact. This impact is created along various steps in the lifecycle, e.g. during the extraction of raw materials or production. This white paper will explore the various stages of a battery's life cycle and how they contribute to its overall Global Warming Potential (GWP).
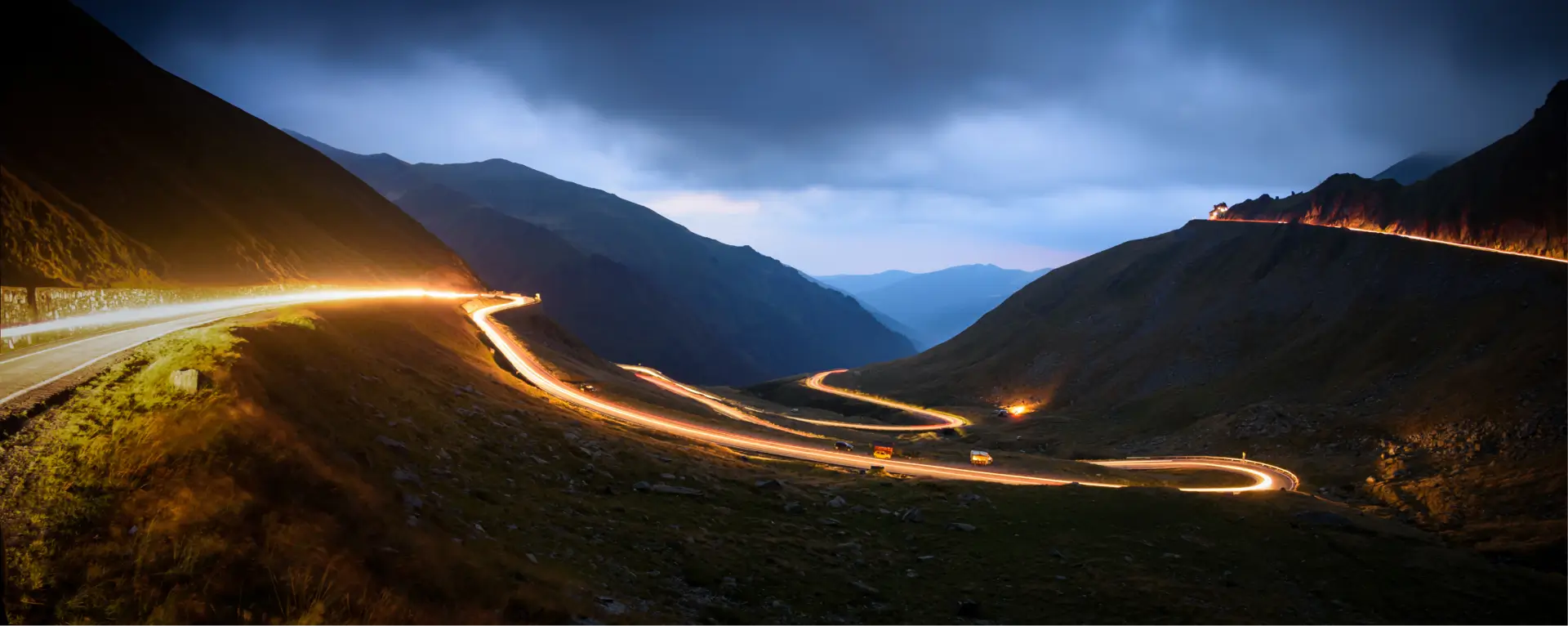
Batteries and their Global Warming Potential: a lifecycle view
Batteries and their Global Warming Potential: A Lifecycle View
.jpeg)
Introduction
Lithium-Ion-Batteries are a key technology for green energy, mobility, and for this reason a key technology to mitigate climate change. However, batteries have a significant environmental impact. This impact is created along various steps in the lifecycle, e.g. during the extraction of raw materials or production. It is heavily influenced by later decisions of how the battery is used not only in its first in-life phase, but also during second life and end of life. It is essential to minimize this environmental impact and keep batteries in use for as long as possible. This can be done by making more sustainable choices at each stage of the battery life cycle.
Battery analytics plays an important role in making these choices. By providing insights into battery performance, aging estimation and modeling, battery analytics can help optimizing battery design and usage and thereby extend the battery life.
This white paper will explore the various stages of a battery's life cycle and how they contribute to its overall Global Warming Potential (GWP) and will discuss how battery analytics can be used to minimize the environmental impact.
Sourcing and Refining
Currently, when trying to assess batteries’ GWP, obtaining accurate data within sourcing and refining of battery raw materials such as cobalt can be challenging due to lack of transparency and unclear boundaries between material refinement and cell production. Furthermore, diverse sourcing and mining practices are adding difficulties. Although primary data on raw material sourcing is difficult to obtain, it still can be concluded that the influence of mining and refinement of the cathode materials has a relatively low impact on the GWP compared to other parts of the battery. In the future, initiatives like the Battery Pass, which is a leading ongoing EU-funded project where TWAICE serves as experts, will make sure that information about the origin and environmental impact is available throughout a battery’s lifetime covering all relevant parts of the battery. Â
Aluminum is a significant GWP contributor with its high share of the whole battery and energy-intensive production. Although the required energy for recycling aluminum is considerably low, the use of recycled aluminum in batteries is unclear due to potential compatibility issues. Differences in alloy grades and other requirements for the aluminum components in LIB may make the use of recycled aluminum unattractive. For this reason, the share of recycled aluminum in batteries is considered low.
Positive, on the other hand, is that materials other than those in the cathode have minimal impact on the GWP of a battery cell. Interestingly, despite forming a significant portion of the cell's composition, the anode paste, electrolyte, separators, and electrode substrates (together 45% of the NCA cell) collectively contribute less than 8% to the overall environmental impact.
Battery Manufacturing
Manufacturing emerges as the primary driver of battery emissions, with cell production demanding significant energy. The resulting GWP is heavily influenced by the plant's capacity, production methods and the regional electricity mix used. Notably, producing cathode powder involves a two-stage process that significantly increases energy demand. For this, the required kilns operate continuously, regardless of production volume, amplifying the GWP impact. This makes the GWP of the cathode material highly dependent on the capacity and throughput of the production facility.
Studies highlight the dependence on the energy mix used in the location of the respective production facilities, showing a substantial increase in emissions when shifting production from regions like East Asia (Japan or Korea), with a cleaner mix, to locations like China, Poland, or India. Considering published LCAs (=Life cycle assessments) the emissions for the cell production compared to an East Asian (Japanese or Korean) electricity mix increase by 5% if the US electricity mix is considered. 59%, 69% and 112% respectively, when productions are located in China, Poland or India instead. Cell production in Sweden just leads to as little as 7% of the emissions compared to East Asia. However, the exact influence of the energy mix on the CO2 contribution often remains uncertain due to big differences considering the assumptions of required energy for the production.
Design Decisions
The materials chosen for cell housing and modules, like plastic or metal, play a role in the GWP as well. While plastic might be preferable for Lithium Iron Phosphate (LFP) batteries, safety concerns often necessitate metal in Lithium Nickel Cobalt Aluminum Oxide (NCA) batteries, highlighting a trade-off between environmental impact and other factors like safety. In this matter the safety issue appears due to a higher thermal instability of NCA. After the very CO2 intensive production of a LIB, LIB don’t emit greenhouse gases during the in-life period, resulting in big savings compared to combustion engines when considering the mobility & energy sector. Therefore, the longer the battery lasts the better it is for the environment. One of the challenges (e.g. for automotive OEMs) when it comes to battery system design is that manufacturers have to provide a battery that lasts for several years but is not oversized, as oversizing implies an unnecessary usage of resources and thus additional CO2 emissions. The difficulty in sizing a battery for such a long period is that the aging heavily depends on the use. To provide a certain performance at the end of life as well, batteries tend to be oversized considering their initial capacity.
To help battery manufacturers designing their systems in the economic and ecological best way, battery analytics can help provide accurate battery simulation through modeling the state of health (SoH) and other metrics throughout a battery’s lifetime and different usage scenarios. Furthermore, reliable predictions of a battery’s end of life (EoL) can be made and the right depth of discharge (DoD) can be assessed to help dimension the battery correctly.
SoH and EoL estimation and prediction are necessary to specify the required capacity throughout a battery’s lifetime considering aging and thereby loss of usable energy capacity. As a consequence, poor SoH prediction results in oversizing the battery to compensate the lack of certainty regarding the battery’s usable energy capacity over its time.
The DoD on the other hand is a main pain point influencing the battery’s cycle life. More insights about the challenges connected with the DoD can be found in the section about In-life.
To conclude, better SoH prediction and DoD assessment helps to size the battery correctly and therefore, the carbon footprint of battery productions can be decreased through material savings.
In-Life
The depth of discharge is a very important parameter which is crucial for the cyclic aging of the battery during its use. For this reason, it is very important but challenging at the same time to define the DoD which best suits a specific battery design. Batteries are aging calendrically the fastest if they have a critical high or low state of charge (SoC). Therefore, producers are limiting the SoC on both sides resulting in shorter cycles which increases the number of cycles and leads to cyclic aging. This issue results in limiting batteries real life operation so that it is possible to use not more than 70-85% of the nominal capacity. With more knowledge about the possible DoD these mechanisms can be avoided, and batteries can be sized more accurately.
Another crucial topic related to the cyclic aging of a battery is the charging strategy. In recent times research mainly focused on reducing the charging time. This parameter is connected strongly with the convenience of driving an electric vehicle which is essential for the transition from combustion engine cars to green mobility. Nevertheless, fast charging harms the state of health of the batteries and therefore strategies are needed which keep a balance between temperature rise, battery degradation and charging duration. This task is particularly difficult because of the strongly non-linear aging of Lithium-Ion-batteries.
Popular charging methods like CCCV (constant-current-constant-voltage) are easy to implement but not optimal in terms of degradation. Newer methods like boost charging, pulse charging or hybrid protocols often neglect battery health in favor of charging time and consequently accelerate degradation. These methods don’t contain any information about the mechanisms responsible for the aging of the battery like the condition of the battery during charging or the battery temperature.
Battery analytics and model-based charging protocols offer more promising methods. They use models to understand how batteries age, resulting in better designed charging strategies. For example, they can use the specific SoH and ScC of the battery to choose the right charging currents to improve charging speed, energy efficiency, aging and capacity fade. SoC and SoH cannot be measured directly but are based on the battery model parameters and state variables. Comparisons between conventional CCCV charging and model-based optimized strategy in terms of lifetime extension resulted in an extension of the service life by 27% compared to the conventional CCCV charging method.
Factors which further influence the battery performance can be separated in manufacturing variations (intrinsic) and environmental factors (extrinsic), collectively known as cell-to-cell variations (CtCV). These variations hurt performance in both the short and long term. In the short term, they can reduce the overall capacity of the pack. In the long term, they lead to uneven aging and different degradation rates in individual cells, ultimately causing the entire pack to reach its end-of-life sooner than it should. Replacing individual modules can extend the system’s life. However, accurate battery health diagnostics are crucial to make it possible to identify which modules need to be replaced and when.
To find any kind of anomaly inside a battery, constant battery analytics monitoring during in-life is a key factor. While traditional, scheduled maintenance is common, predictive maintenance monitors batteries’ actual health, allowing for targeted intervention before issues arise. This approach, already used in other industries, is significantly extending the life of energy storage for example, as it can address individual cell and module variations within a system and prevent unnecessary breakdowns, schedule necessary maintenance activity while maximizing the periods in between.
Second Life
While electric vehicle batteries typically have reached their EoL of their original purpose once their capacity degrades to 70-80% of their initial value, they still hold significant potential for second life applications. This approach leverages the remaining capacity in these batteries for alternative uses, offering several environmental and economic advantages.
Firstly, by reusing batteries instead of immediately recycling them, the significant environmental impact of manufacturing new batteries, including resource extraction, further production and related emissions, is avoided or delayed. This extends the overall lifespan and value of the battery, while sharing its carbon footprint across both its initial use in an EV and its second life application. In this way it is offering a compelling strategy for optimizing resource utilization and minimizing the environmental impact of lithium-ion batteries in the long run.
Three main approaches for a second life use are:
- Remanufacturing: This involves repairing and refurbishing batteries for their original automotive use. It requires extensive testing, disassembly, replacement, and reassembly to meet the original specifications. However, not all battery modules might be suitable due to degradation, and only a portion of the original pack might be usable. Remanufactured batteries can then serve as spare parts.
- Repurposing: This involves reconfiguring batteries for less demanding applications, typically stationary uses like energy storage in homes or businesses, or other electric vehicles like scooters or forklifts. Repurposing also often involves testing, disassembly, and replacing damaged units, but additionally requires restructuring and new hardware/software for the new application.
- Reusing: A battery that has been subject to re-use or preparation for re-use. Re-used means any operation by which batteries or components that are not waste are used again for the same purpose for which they were conceived (Waste Directive, Article 3(13) referenced in the EU Battery Regulation, Article 3, 2(a))
Important for second life is the battery’s accurate state of health and other parameter assessment. Factors like voltage, current, capacity, size, shape, and temperature range are important to screen before further use is defined. Moreover, the state of health (SoH) and remaining useful life have to be determined in order to make optimal decisions for the second life. The SoH assesses capacity loss and internal resistance increase to ensure reused batteries can meet the application's power and energy demands. Furthermore, RuL is important for the prediction of the battery’s end-of-life. This is crucial for maximizing its second life value but challenging due to individual aging and usage history. Battery analytics software, however, does provide the solution to these challenges.
Conclusion
As battery demand increases, the need for accurate battery analytics becomes crucial to lower a battery’s CO2 footprint. But also, the growing movement towards CO2-neutral battery production offers a promising solution to establish more efficient production facilities to lower emissions during production phases. Additionally, developing batteries with higher energy density and optimizing module and pack design can further reduce the GWP. However, short-term gains might be offset by battery production concentrating in regions with fossil fuel-based electricity mixes in some regions.
Better SoH prediction and DoD assessment can help size the battery accurately, reducing the carbon footprint of production. In-use factors like charging strategy and cell-to-cell variations also affect the battery performance and lifetime. To observe such mechanisms and increase the life span, anomaly detection, optimal model-based charging and predictive analytics are promising techniques to improve battery health. After the use in electric vehicles, the batteries can be given a second life in other applications such as energy storage to stretch emissions over a longer period of time.
Summarizing, the carbon footprint of batteries is a complex issue influenced by various factors throughout the battery’s lifecycle. Transparency and standardization in data collection and assessment are crucial for accurate estimations and future improvements which TWAICE is addressing through close collaboration with initiatives such as the Battery Pass project. While challenges remain, advancements in design, manufacturing, and especially energy sources offer promising potential for reducing the overall GWP of batteries. With battery analytics software and services, TWAICE further contributes to lowering the overall GWP of batteries through offering its customers a significant CO2-reduction potential by adapting the TWAICE Battery Analytics Platform and battery development solutions, thereby extending batteries’ lifetime and providing the decision ground for optimal battery design and usage during a battery’s first or second life.
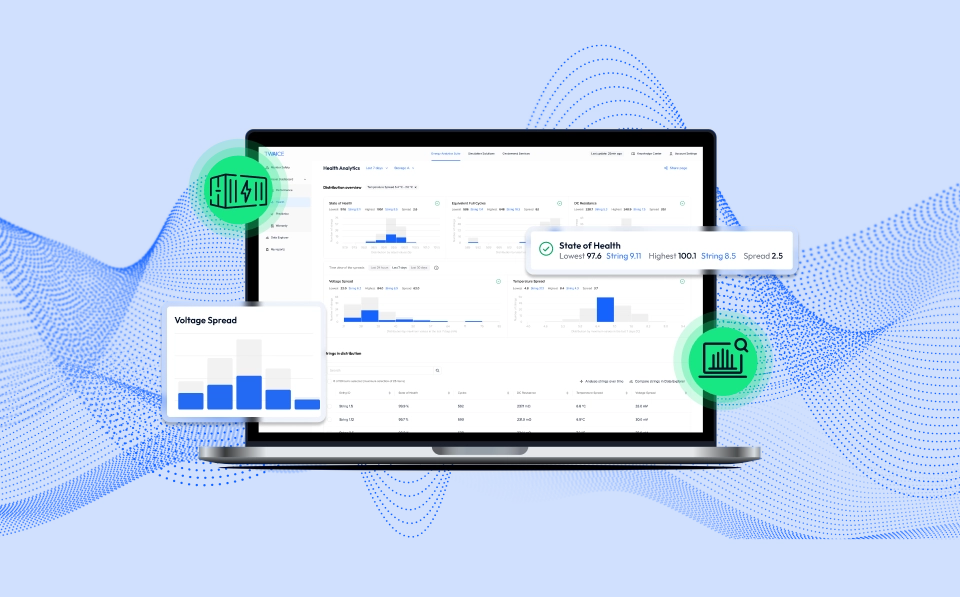
See TWAICE Energy Storage Analytics in Action
Sign up for the next live group demo and learn how TWAICE can transform your BESS operations. In just 30 minutes, you’ll get a demo of key features and use cases, and engage with our product experts for a live Q&A.
Related Resources
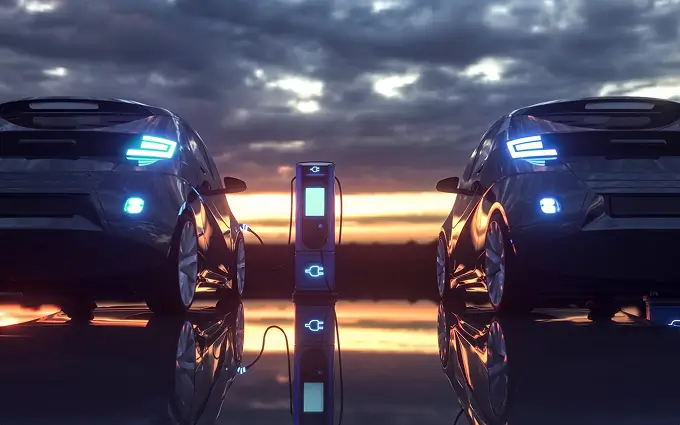
Modeling Lithium Plating Conditions Over Aging for Safe Li-Ion Battery Fast-Charging
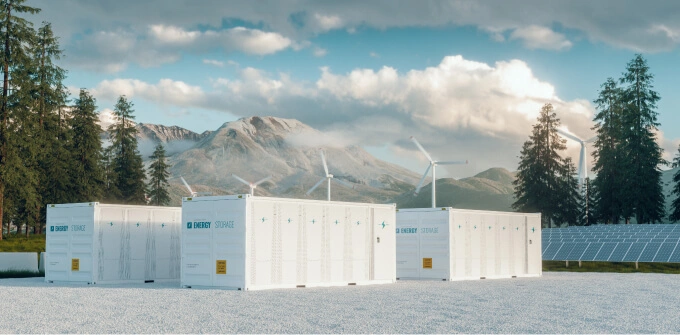
Overcoming Challenges in SoC Estimations for LFP Energy Storage Systems
(1).webp)